Rick Gaitskell and I first met over lunch in Deadwood, South Dakota, back in the summer of 2012. An astrophysicist who had quit investment banking because he found the mathematics of finance too basic, Gaitskell had just emerged from an underground laboratory that was once among the nation’s richest gold mines. He was now sitting across from me and talking, quite literally, about everything in the universe.
He swooped in great conversational arcs from his English boyhood to the best method for extracting a single recorded voice from a babble of background noise, from the nature of weakly interacting massive particles (WIMPs) to the shocked reactions from his Brown University students when they first hear his profane language in the classroom (“I don’t think it’s Tourette’s”). He described a computer program he had written for his cell phone, which allowed him to measure the g-forces exerted on the core of a dark-matter detector as the device was moved underground, since its wires were so hair-thin that the mildest acceleration might break them. He veered to the news that a few days earlier had held the scientific community spellbound: the Large Hadron Collider, at the European Organization for Nuclear Research (CERN) in Geneva, had finally pried loose the Higgs boson — the “God particle,” so called because it gives other particles mass and therefore allows all the matter we see to be matter — and disclosed its irreducible splendor.
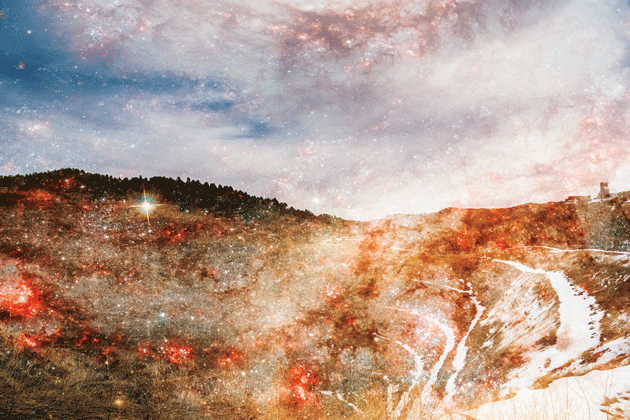
Composite photographs by Tatiana Gulenkina. The Open Cut at the Homestake Gold Mine, Lead, South Dakota, (Tatiana Gulenkina) and the Whirlpool Galaxy (NASA)
Physicists had theorized the Higgs boson fifty years ago, as the linchpin that would complete the Standard Model of elementary particles. As Gaitskell noted, the people who had devoted their careers to the Higgs were therefore older than the theory itself. Yet even as the scientific community was pooling the immense resources of money and technology that would allow it to free the Higgs, the Standard Model itself was fracturing under the tectonic pressure of new discoveries.
No wonder Gaitskell periodically leaned over the table and placed his hands on either side of his dark-haired head, as if he were trying to keep ideas from bursting through his skull. After all, the purpose of Gaitskell’s subterranean dark-matter detector was to find a missing fraction of the universe: perhaps one quarter of the entire cosmos. He was talking about a revolution in thought as great as the one that changed gravity from a straight-line force into a bend in a continuum and revealed time and space as siblings. It all evoked the end of the Ptolemaic system, repeatedly tweaked to save the appearances of planetary and stellar movements — until Copernicus’s Earth-decentering elegance could no longer be denied. Gaitskell and his colleagues are approaching the revelation of a new order, a new universe, in which even light will be known differently, and darkness as well.
Gaitskell conducts his research at the Sanford Underground Research Facility (SURF), in the adjoining town of Lead, South Dakota. Located at almost the exact geographic center of the United States, Lead is an improbable place, its houses rammed into precipitous inclines, its streets shooting upward, the whole assortment perched a mile above sea level. Businesses cling to the sides of Main Street, which, as you turn off U.S. 85 at the western end of town, drops like a roller-coaster track and then winds past real estate offices and restaurants and a mining museum and a theater and finally, on your left, the immense pit of the Homestake Gold Mine’s Open Cut. Just beyond is the pit’s visual and actual inversion, the mountain of tailings removed from it, stair-stepping into the sky. Pit and tailings seem far too large not to be innate to the landscape, yet you may sense a miscue in perception as you drive by, which you will shrug off as you leave town to head toward Deadwood’s clanging gambling halls or the RV-jammed highway to Mount Rushmore and the Crazy Horse Memorial, fifty miles south.
Lead’s houses are a mixture of the proud and the ramshackle, the former built by men made rich on Homestake’s gold, the latter by miners who weren’t about to waste money on fretwork and frills when the rock under their foundations might be removed. Many miners built on land actually owned by the mine, with ninety-day leases and no guarantee the Open Cut wouldn’t reach their doorsteps before those leases expired. But whether you leased land and built just-good-enough, or bought and built with gables and wraparound porches, you shared the certainty that the mine itself — that relentlessly expanding and deepening cavity — would last.
George Hearst came to Lead from the California gold fields in 1877, the year after George Armstrong Custer met his demise at what the Lakota call the Battle of the Greasy Grass. Hearst was a mining genius. In the Homestake he saw “such a deposit of ore as I never saw or dreamt of before” — so he gladly paid $70,000 for the claim. It had been just three years since Custer had led a military expedition through the Black Hills, or Paha Sapa, of the Great Sioux Reservation, seeking to confirm rumors of gold in its streams. By the time Hearst showed up, thousands of miners were already digging into lands ceded by treaty to the Lakota, because even if the Constitution called treaties “the supreme law,” putting them on an equal footing with the Constitution itself, nothing, legal or otherwise, trumped gold.
Long before geographers fixed the nation’s center at Lead, the town’s residents believed they were there already, and they weren’t wrong. Homestake was the longest-listed mining corporation on the New York Stock Exchange, and Lead was that paradox of authentic America: an immigrant town of dozens of cultures and tongues where men seeking opportunity communicated in the language of work. By 1909, the city boasted a thousand ore-crushing stamp mills making so much noise that when managers locked out the miners to quash a nascent union, a local paper noted that “you could even hear the dogs bark.” Later, the corporation kept the union at bay with a free hospital, a recreation center, and parks — and that inexhaustible future.
The reality of Homestake exceeded anything even Hearst could have dreamt: it produced 41 million ounces of gold, worth almost $47 billion in today’s market. The invisible, underground portion of the mine just kept going, 8,000 feet down, with 370 miles of horizontal tunnel, or drift — comparable in length to the New York City subway system. Along with the Open Cut, it was far too large to seem a mere human artifact or despoliation of nature. So of course Homestake’s closure, in 2002, was met with disbelief. Even after employees had received their last paychecks, some people claimed it was a bargaining chip for lower wages. Geologic features don’t shut down. They are.
After 125 years, in fact, the mine still contained plenty of ore. Its failure was predicated on its own boundlessness. A mine is no inert hole in the ground, but is as technologically sophisticated and expensive to operate as a skyscraper. Consider the difficulty of that most basic thing — surveying and running a drift a mile through the earth with absolute accuracy. Consider the heat at 8,000 feet, more than a hundred degrees, and the cost of ventilating 7,700 subterranean acres, and the frangibility of copper wire, which will break under its own weight if dropped thousands of feet down a shaft and must be reinforced with steel cable and periodically attached to the walls. Add the cost of operating those hydroelectric plants whose water rights Hearst fought so tenaciously (and with borderline legality) to obtain. Consider the wear and tear on equipment that crushes tons of quartz ore every day, and the water seeping from the rock walls of the mine, a small river that had to be pumped out at a monthly cost of $250,000.
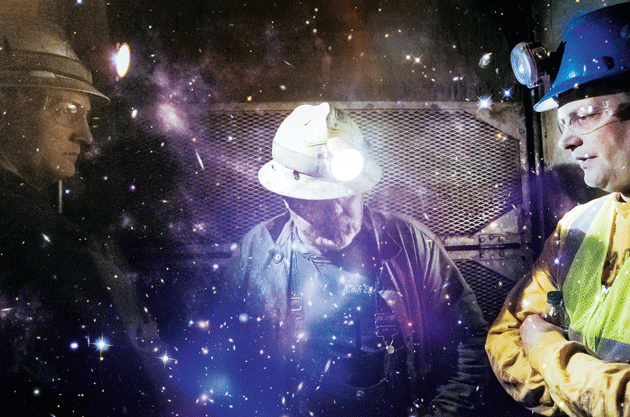
SURF workers in the elevator cage at the Homestake mine (Tatiana Gulenkina) and colliding galaxy clusters (NASA)
Homestake’s best veins produced half an ounce of gold per ton of ore, and by the end of the 1990s, the precious metal was fetching less than $300 per ounce. The mine was barely breaking even, and it grew more costly with every lengthened drift and deepened shaft. If some citizens disbelieved its closing, civic leaders recognized a business decision and a disaster: 450 people out of work, Lead’s economy wrecked, real estate values destroyed, mineral severance taxes gone. Lead would be left with the setup to a bad joke: What do you do with an 8,000-foot-deep hole in the ground?
But there was an unexpected punch line. Another story had germinated in the mine, a story about astrophysics, a Nobel Prize, and subatomic particles. When Homestake became a hole in the ground, this other story ballooned with remarkable energy. The mine, born out of Custer’s search for a bright, rare element in the earth, now flipped inside out to face the stars. It became the locus of a far more difficult and expensive search, for substances not rare at all but infinitely more elusive.
During the late 1960s, while Homestake was in its prime, a chemist named Ray Davis installed a 100,000-gallon tank of perchloroethylene — a dry-cleaning fluid — 4,850 feet down in the bowels of the mine. He wanted to study the sun’s neutrinos, and had the notion that the best way to accomplish this was to filter everything else out.
Understanding what Davis was doing requires some scientific history. As early as the 1930s, scientists knew that in the process of radioactive decay, an atom’s nucleus will release an electron. They were puzzled, though, by variations in the electron’s energy level. The law of conservation of energy dictates a mathematical tidiness, whereby all such differences had to be accounted for. The Swiss physicist Wolfgang Pauli suggested a solution: Perhaps the electron was accompanied by an undetected “ghost particle,” which was more energetic when the electron was less so, and vice versa. This particle came to be called the neutrino, or “little neutral one.” Pauli thought it might never be detected because it would barely react with matter.
Subsequent breakthroughs refined Pauli’s idea. James Chadwick, a British physicist, discovered that atomic nuclei contain not only protons but neutrons, which turn into protons by releasing an electron plus that missing bit of energy. The German physicist Hans Bethe worked out the idea that stars are powered by nuclear fusion, and though his research didn’t focus on neutrinos, scientists suspected that there might be enormous quantities of them emitted by the sun. All these discoveries came during the 1930s, but it required the invention of nuclear power plants, which absolutely spew neutrinos, for Frederick Reines and Clyde Cowan to finally confirm the existence of the particles in 1956.
By the late 1960s, Davis knew that if the reigning theories of solar fusion were correct, trillions of neutrinos per square inch were passing through Earth every second. To test those theories, he would attempt to measure the size of the sun’s neutrino stream deep underground, where the mile of rock would filter out radiation from other sources, most notably from the atmosphere. The neutrinos would be like great schools of minnows flashing through nets designed to catch sharks. The net would stop the sharks, and though Davis wouldn’t catch the minnows, if he could get a few to bump their noses on knots in the net, he could count the bumps.
The atoms of chlorine-37 in Davis’s perchloroethylene tank functioned as those knots. Every time a neutrino struck such an atom with sufficient force, it would cause a neutron in the nucleus to turn into a proton, transforming the atom into argon-37. Davis could monitor the tank’s quantity of argon-37, which would allow him to calculate the size of the neutrino stream. It was a marvelously designed experiment, the essence of science, with just a single meaningful variable: the number of neutrinos striking the target.1
Davis expected a fairly straightforward result: a confirmation of solar fusion as worked out by the theoretical physicist John Bahcall. Instead, he encountered one of the most mind-bending puzzles in the history of physics — so intransigent that it earned its own name, the “solar neutrino problem” — and changed the methodology of astrophysics from gathering light to eliminating noise, from greater seeing to more sensitive listening. The mountaintop telescope perched in thin air didn’t diminish in value, but its metaphoric opposite, the hole in the ground, dramatically inflated. The deeper the hole, the more it could filter, and one of the deepest holes on the planet was the Homestake mine.
I visited SURF a week before my conversation with Rick Gaitskell, arriving at the nation’s newest and arguably most important subterranean laboratory through the same gate that miners once used and parking my car in the immense lot overlooking the Black Hills. I found the SURF offices, signed a liability-release form, and got a hard hat with a mining light and a W-65 respirator in case my air supply was compromised. Then I and a handful of other reporters followed Bill Harlan, who was SURF’s communications director at the time, into the elevator cage. When the cage carried thirty-six miners packed chest-to-back, or the parts out of which to construct, in the shops below, the immense trucks that once roamed the drifts, the ride down to 4,850 feet used to take three minutes. Now it took ten.
Harlan, a cheerful balding man, joked about being the worst gold miner in the history of Homestake. Meanwhile the cage operator, also a former miner, directed his colleagues to release the safety catches. The cage lurched up and the catches clunked inward. We were now suspended from cables with almost a mile of air beneath us. Banter among my fellow passengers dwindled. The dark timbers of the Yates shaft, built in the 1930s, began to slide smoothly upward past the open grillwork, dripping water. As we went down, the quantity of water increased, until we were standing in a tiny, boxed-in rainstorm. Every now and then a black hole blipped past, a tunnel bored into the earth, a blink of vacancy and depth where the cage had once stopped to let men out.
It stopped for us at the “forty-eight-fifty,” once the main staging area for the mine, now SURF’s Davis Campus. From here, men used to descend to other drifts, spreading out to mine faces some three thousand feet deeper. Wind, the product of the mine’s immense ventilation system, roared down the shaft when the cage’s doors opened, blowing the falling water against our backs as we left. I felt a great sense of underground: steel-grate walkways, the smell of exposed rock and earth, a small yellow electric shuttle at the edge of the dark drift that led to the Ross shaft, a quarter of a mile away.
Harlan led our group around a corner, and the sense of being underground dissipated. The tunnels here were sprayed with shotcrete, rocky foam reflecting the bright light. Then the walls became vertical, and we were walking on smooth interior floors, passing doors that led to laboratories. Graduate students sat at tables lined with laptops. We could have been in a college-classroom building, but it was no typical lesson we were about to learn. Instead, we would confront the possibility that the matter we know is only a tiny sliver of what is really there — or here, since if physicists are correct, stuff we can’t possibly sense is passing right through us, not just neutrinos but matter so unknown it is only a theory and yet so massive that it may constitute far more of the universe than does the matter we can see and touch. We would be asked to consider that all of us are a minimal presence, wisp and froth passing through a dark sea, sieves made of a rare gossamer — as is Earth, as is the sun, as are the compressed and golden cores of all the stars.
There are four known forces in physics: the strong nuclear force, electromagnetism, the weak nuclear force, and gravity. The relationships among the first three of these and the particles with which they interact constitute the Standard Model, which is one of the twentieth century’s greatest intellectual achievements. In this model, “baryonic matter,” cemented together by the strong force, is the visible matter of the universe. When Dr. Johnson kicked his rock to refute Bishop Berkeley’s notion that the world might be only an idea, his sore toe was a testament to the tenacity of the strong force, as is the staggering amount of energy required by CERN’s Large Hadron Collider to break atomic particles into such irreducible components as quarks and bosons.
The neutrino is affected only by the weak force, which means that its interaction with baryonic matter lies somewhere between negligible and nil. To a neutrino, what we regard as visible matter is nearly transparent. Between the moment Dr. Johnson drew back his leg and the moment his foot met the rock, an almost endless stream of dreamlike neutrinos passed through his toenail. One hundred trillion of them pass through each of us every second and continue right on through Earth and would travel without hindrance through 100 million miles of solid lead.
Two facts about the neutrino’s nature are crucial for understanding the enigma of Davis’s Homestake experiment — and the ways in which that experiment eventually shook up the Standard Model. The first is that the mathematics of the model require the neutrino to have no mass. The second is that the neutrino comes in three distinct “flavors.” When Davis measured the number of argon-37 atoms in his tank, he found only a third as many as Bahcall’s theory predicted. No matter how many times he repeated the procedure, he got the same result. It was a case of an elegant and precise experiment not supporting an impeccable theory. For three decades, the solar neutrino problem remained unsolved, in spite of increasingly sophisticated underground experiments in Canada, Japan, and Europe.
Finally, in 2001, researchers including Kevin Lesko of Lawrence Berkeley National Laboratory demonstrated that the neutrino is a kind of Trinitarian particle, three neutrinos in one, each distinct at the quantum level yet able to become the others. As the sun’s neutrinos traveled 93 million miles to Davis’s tank, they changed from one variety to another, a phenomenon called neutrino oscillation. Only one of these varieties caused radioactive decay in chlorine-37. The theory of neutrino oscillation turned out to be so powerfully suggestive that in 2002 Davis shared the Nobel Prize in physics for opening the gates to its discovery.
The language I have been using to describe neutrino oscillation is approximate at best. Boris Kayser, a particle-physics theorist at the Fermi National Accelerator Laboratory, told me that any metaphor automatically misrepresents neutrino oscillation, the quantum world being so different from the macro one. Oscillation does, however, require the neutrino to have mass — which sends a tremor through the Standard Model. And in math and physics, a tremor is more or less an earthquake. The neutrino, which had nestled comfortably into the family of subatomic particles, its kinship clear, suddenly seemed an erratic and bizarrely behaving adoptee with unknown origins. Accommodating it to the Standard Model would require more than a few minor fixes. It might in fact require an entirely new system.
Not many years ago, popular treatments of science claimed that physicists were on the verge of linking relativity to quantum theory — or linking the Standard Model to gravity — which would allow them to explain everything in the universe with one set of equations. Neutrino research has ended that assumption. New discoveries, both theoretical and experimental, are now rampantly possible, and some of the most profound questions may be answerable. What goes on inside supernovas? Given the powerful laws of symmetry, why did the universe not destroy itself during the Big Bang with equal parts of matter and antimatter packed together? All these questions are emanations of Davis’s experiment, all their potential answers bound to the kind of laboratory he pioneered.
Homestake closed the year after the solar neutrino problem was solved. By that time, the United States already had more scientists engaged in neutrino research than any other nation. Yet our underground labs were inferior to those in other countries. Homestake’s closure, while a disaster for the citizens of Lead, looked like something else to American physicists: a rare opportunity to address a major deficit in U.S. scientific infrastructure. All they had to do was find someone to buy the mine.
Rick Gaitskell may find the mathematics of finance simpler than the mathematics of physics, but nothing I learned about neutrinos surpassed in complexity the brain-teasing political and financial machinations by which Homestake Gold Mine became SURF. The transformation started at the federal level, as the National Science Foundation (NSF) and the Department of Energy (DOE) wrestled over their respective roles. Meanwhile, Barrick Gold, the Canadian company that bought Homestake’s resources, shut off those $250,000-per-month pumps in 2003, and the mine began to fill with water. Mike Rounds, the governor of South Dakota at the time, suggested that the state simply appropriate the mine, but Barrick feared that such a transfer would still leave the company open to lawsuits. Rounds then proposed the creation of an independent agency that could buy the requisite liability insurance, shielding both the mine’s former owner and the state itself.
Thus was born the South Dakota Science and Technology Authority. In 2004, the state legislature funded the agency with $14 million, and it added another $20 million the following year — considerable sums for a state with a population the size of San Francisco’s. In addition, T. Denny Sanford, a local philanthropist who had made a fortune in credit cards, contributed tens of millions of dollars in private funding. When the NSF and DOE came back on board, a unique, acronym-intensive federal-state-private partnership was born.
The Open Cut remains a powerful visual reminder of the convoluted history and tangled motivations on which this bright, cooperative dream of science is being built. If you walk from SURF to Main Street in Lead, you are allowed a view of the Open Cut that drivers, their feet on the brakes and their eyes on the plunging road, are prohibited. The ledges of the pit swirl conically downward, narrower and narrower, through striations of deep brown and tan, with red and purplish hues. The Open Cut has the shape of an immense whirlpool, flash-frozen and forever stilled. Or it has the shape of a human ear, the skull of the earth tipped up and the sounds of the universe pouring in.
You cannot see the bottom of the Open Cut from the SURF parking lot or from the viewing platform near the visitor center. From the platform, however, you can see cavelike holes in the cut’s sides, and were you a stranger without knowledge of the place’s history, you might daydream of descending those sheer walls on ropes, to enter those caves and find evidence of ancient existences — bones, shards, stone tools — or crystal formations begun in floods that rocked the earth before human beings stood upright upon it.
Yet even the uninformed eye would find something too regular and purposeful about those ridges circling downward, and something too squared-off about those cave entrances, which lead to drifts in the underground portion of the mine. If the pumps ever stop churning for good, the seeping water may reach the level of those drifts, and the Open Cut will become something else entirely: a small, deep jewel of a lake, or an environmental disaster.
There is beauty to the Open Cut, but of a ravaged, mournful kind. The place rebuffs perceptual definition and oscillates in the eye. At one moment it is a marvel and astonishment, a landscape that suggests how much human beings are forces of nature, equivalent to millions of years of wind or water. The next moment it’s a place cored and destroyed, a scar that will never heal, and human beings are not forces of nature but forces against it. The next moment still one can think that the earth has no prejudice toward or against any state or condition, but creates its own deserts, makes its own wastelands, and some wastelands are beautiful and others are not.
K. C. Russell is SURF’s cultural-diversity coordinator. I visited him in his office in the brick building that was once the mine’s headquarters. Russell invited me to sit, then waited for me to begin our conversation. He emanates an atmosphere of patience and intensity, friendliness and focus. When I asked him to describe his job, he started by telling me that he is mixed-blood Lakota and lives the different histories his position asks him to represent. He has a background in science. He drives up to 40,000 miles every year to speak to tribal, community, and school leaders. He oversees the lab’s partnerships with nearby colleges, and his office administers scholarships for students to attend summer classes and to intern underground.
SURF also sponsors an annual Neutrino Day festival, which I attended in 2012 along with nearly a thousand other people. The excitement at this event was palpable, the questions from the audience astute. In the age of the Internet and the Discovery Channel, people were flocking to scientific lectures as they had during the Romantic period, when scientists were celebrities. I had the distinct impression that per capita understanding of astrophysics must be higher in western South Dakota than anywhere else in the world.
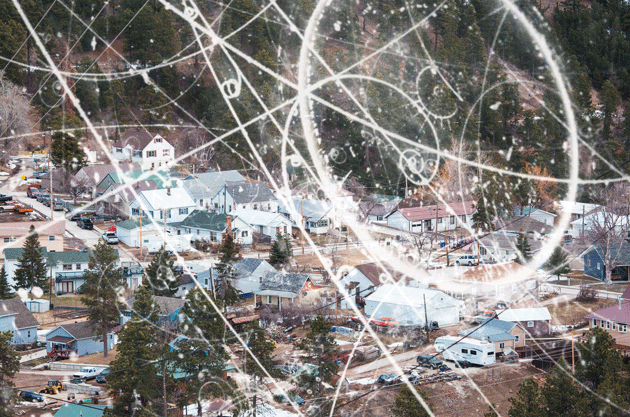
Lead, South Dakota, (Tatiana Gulenkina) and neutrino tracks (© Dan McCoy/Rainbow/Science Faction/Getty Images)
Yet Russell’s work concerns more than the bright face of education, and he quieted when I asked him about the “cultural” part of his title. Cultural issues at the lab are as complex as they were when Lead was a city of European and Chinese immigrants. The Paha Sapa are the center of the Lakota cosmos, a fact it is Russell’s job to honor. Those tons of rock brought up from underground to make space for the lab must stay in the Hills. The water-treatment plant uses a microbe native to the region, since the water’s purity is a cultural as well as an environmental concern.
Russell leaned back in his chair, physically relaxed but intellectually forceful, as he mentioned the 1868 Fort Laramie Treaty and Custer’s presence in the Paha Sapa. I knew what he was referring to and understood its weight. If one man can serve as a lens for the disenfranchisement of an entire people, that man, for the Lakota, is Custer. His sins against them are numerous, but as great as any is that by entering their land, he broke a treaty that was meant to last forever.
Tourists who make their way northwest from Lead to Devil’s Tower may find a small historical marker along the highway. Those who stop will see a very literal landmark: tracks scored into the earth by the steel-rimmed wheels of Custer’s two hundred wagons — another open cut that has not healed. When Custer reported the discovery of gold, the government gave up trying to stop the swarms of settlers entering the Black Hills, and miners illegally staked claims to land still ceded to a people who did not believe in private ownership of the earth.
The government forced the abrogation of the treaty — until 1980, when the Supreme Court overturned that abrogation. But even the Supreme Court could not undo a century of development, and its proposed compensation was notably mingy: $106 million, derived from the market value of the land in 1877 ($17.1 million) plus 5 percent interest over 103 years. The Sioux Nation refused settlement, demanding the original terms of the Fort Laramie Treaty. With interest, Russell said, the unclaimed money has now grown to more than a billion dollars.
Russell asked me to consider the value of 41 million ounces of gold in today’s market. He didn’t provide the answer, but the price of gold the week I interviewed him was $1,650 per ounce, and later I worked out the $64 billion imbalance. Russell believes in the future the lab offers, its promise and potential, for science and for people of all ethnicities. Still, it is the massive past, sometimes invisible but always passing into and through the present, that defines the job he does.
Back underground, Harlan shepherded us into the laboratory area and down some yellow steps to a welded-steel tank some thirty feet in diameter and twenty feet high. This tank would be filled with 71,600 gallons of deionized water surrounding a six-foot-tall thermos of liquid xenon held at –160 degrees Fahrenheit. The water would filter out almost all the radiation the rock had not; the xenon would be purified to the point that it was virtually radiation-free. Thermos, tank, and the surrounding earth constituted the Large Underground Xenon detector (LUX), designed to find those theoretical bits of dark matter known as WIMPs.2
SURF is at the frontier of the nation’s neutrino research. It is running an experiment to determine whether a neutrino can be its own antiparticle — the answer to which may tell us why the universe exists at all — and has planned a study of neutrino oscillation. But with LUX, Gaitskell and Tom Shutt, a physicist who now works at the SLAC National Accelerator Laboratory, are pursuing another, perhaps related mystery. In the 1960s, around the time Davis was setting up his tank of dry-cleaning fluid, scientists noticed that stars at the edges of our galaxy seemed to be orbiting faster than they should be, given the galaxy’s measurable mass and gravitational energy. There was only one reasonable explanation: the galaxy had to be more massive than it appeared.
Physicists called this unknown mass dark matter. Additional observations of the way light bends around distant galaxies supported the earlier data, indicating that the matter we can see — in stars, nebulae, and dust clouds — is only 4 to 5 percent of what the universe actually contains.
This statistic startled even the physicists. It had taken decades for the concept of a vacuum to replace the idea of an ether between the stars — and now that vacuum apparently thrummed with substance. There were attempts to modify Newton’s theory of gravity to explain the enigma, but a preponderance of evidence now supports the reality of dark matter. In fact, astrophysicists believe that without dark matter, stars could not have clustered into galaxies.3
As with the neutrino, then, dark matter may help us understand the very conditions of our existence. Competing theories give dark matter different forms. Gaitskell and Shutt are betting on particles that would fit a complex set of equations linking Big Bang conditions to the weak force and the universe’s missing mass — a concatenation called the WIMP miracle, since the mathematical alignments are almost too perfect to be true, or too perfect not to be. The WIMPs the two men hope to detect will react only weakly with baryonic matter. They will, however, disrupt the atoms of noble gases such as xenon if they hit them directly. This disruption should displace a single photon and a single electron — hence the delicate design of LUX’s core.
Surrounding the thermos of liquid xenon in the detector are photo-multiplier tubes so sensitive they can detect that single photon, while an electric field will draw the electron to the surface, where it will emit a flash of light. By measuring the time and distance between these two flashes, the investigators will be able to pinpoint where the collision between the unknown particle and the xenon atom happened. Events in the center of the thermos will be prime WIMP candidates, while those closer to its edge are more likely to be radioactive contamination.
When Shutt and Gaitskell finished explaining the device, the reporters around me began asking questions. I removed myself. I had grown up on Carl Sagan’s wide-eyed stare and his “billions and billions of stars” and was accustomed to think of the universe as magnificent in its vastness and indifference. I’ve knelt with my children on cool nights and invited them to peer through a telescope’s eyepiece at the smudged shapes of galaxies. But the universe the LUX detector is set up to discover is something beyond number. “Billions” is an attempt to limit and name what’s there, but dark matter, while not the same as the Greek notion of Chaos, is reminiscent of it — a formless ocean through which forms move, and which moves through them.
To visit Wind Cave National Park in the southern Black Hills, which the Lakota traditionally regard as their people’s point of emergence from the underworld, you walk down metal steps into the earth. The guide turns out the lights and invites you to hold out your hand and bring it slowly to your face. Your hand touches your face and still you do not see it. In the virtually photon-free darkness of Wind Cave, you see as well as a cat or lemur would — not at all. But the darkness Gaitskell and Shutt speak of is of another kind altogether: darkness as substance and presence, not absence. This darkness may turn out to be both far more and far less tangible — because it redefines tangibility — than any religion, myth, or comic book has imagined darkness to be.
In The Structure of Scientific Revolutions, Thomas Kuhn says that science is a product of ancient Greece. Other cultures, while devising ways to advance knowledge, did not do so in the particular way Kuhn defines as scientific. It doesn’t take a Kuhnian, however, to see that science is pinned to culture. Certainly there was a time when the scientist made his discoveries alone, communicating with a small coterie of like-minded people, but since at least the early nineteenth century, when the great academies of science garnered support from their governments, science has been inextricable from commerce and politics.
Napoleon took scientists with him to conquer Egypt and later sponsored research for finding ways to extract sugar from beets in order to undermine a British blockade. The great Augustin Fresnel was asked to invent a better lighthouse lens, which saved so many lives that it may have been more important than his essential work on double refraction and light-as-wave. At the very core of the twentieth century is that confluence of science and politics that lit up the New Mexico desert and poured a culture’s resources into what had been an esoteric science, forever changing war, medicine, and energy. The great churning, scraping, buying-and-selling engine of capitalism that made George Hearst possible also made possible the pure science now taking place in his mine, providing the luxuries of excess energy and wealth that allow people to seek knowledge with no immediate use.
When I asked Kevin Lesko why we should pursue research into neutrinos and dark matter, he compared it to electricity in Ben Franklin’s day. Franklin never imagined that the “fluid” he studied would have an actual use. Lesko’s answer has two facets: on the one hand, science is not motivated by utilitarian concerns; on the other, science leads to utilitarian wonders that we cannot predict. In either case, however, science unmoors us by its very nature, which demands that it leave its own past behind, no matter how assured and comfortable, if new knowledge indicates it should so be left.
No doubt these new investigations will unmoor us, too. Neutrino research strikes me as wizardly and glittering, intellectually deep beyond my plumbing and yet almost Tinkerbellish, moving in a cloud of sparkles, ready to answer questions of magic and delight. How did this lovely stuff we’re made of, this too too solid earth, this bright sun and luminous moon and water we bring to our lips with hands or crystal glasses, manage to spark itself into being? Without that ghost particle, Boris Kayser told me, there would be no fusion and no sunlight.
Dark matter leaves me with different feelings, but not the simple ones the words imply. “Dark matter,” after all, is only a name for what we do not understand clearly enough to give another name. For so many centuries, we have believed that science would encompass more and more knowledge until it encompassed all. And then, in a breath, we discover that what we thought was all is only 5 percent. It may be that the remaining 95 percent is amorphous, and that we are not necessarily missing most of the information the universe contains. Nevertheless, the long, amazing trajectory of scientific thought, which has brought us so far and has felt so much like a heroic journey, can seem, in the face of our newfound and vast ignorance, like a few feeble steps taken under the immensity of an unknown sky.
On October 30, 2013, Gaitskell, this time with Daniel McKinsey of Yale, spoke to another gathering about the LUX detector. This group included the governor of South Dakota and NSF and DOE officials, with scientists from around the world listening in via the Web. LUX had finished its initial run and had just moved dark-matter research to a place it had never been before.
The device, Gaitskell and McKinsey reported, had not found dark matter. Rather, it had nullified earlier data hinting at dark matter’s discovery, thus proving that the search had to be taken to newer, deeper levels. There are scientific successes that can look like failures to nonscientists, and this was one of them. The LUX detector’s lack of response, its complete preservation of the quiet built into it, was the source of Gaitskell’s excitement. You might even call it glee. For twenty-five years he had been searching, and now, though he hadn’t found what he was looking for, he had mapped an area where looking was useless — and so had narrowed the territory that remained.
For 110 days, Gaitskell and McKinsey had been running LUX, calibrating and recalibrating and cleaning it of all radioactive noise, and for 85 days it had worked at full function. They and their team had pored over 1.5 million pages of data (“like reading War and Peace every day for a year,” Gaitskell said), in conditions so emotionally fraught that, as he put it, “we worried ourselves into ecstasy.” Of 84 million possible events that the detector recorded, they had narrowed the data down to 160 worth examining — and none of them, none at all, turned out to be definitive.
To illustrate, they showed a graph of mass-and-wavelength coordinates. Where other detectors in other experiments had recorded potential WIMPs, the LUX graph was as white as a map of Antarctica. According to earlier data, LUX, which is twenty times more sensitive than any dark-matter detector ever made, should have recorded 1,550 events in that space. The graph should have looked like flyspecks on old siding, or an advancing cloud, or, at the best and most exciting, a thin, dark, clean line within narrow, precise parameters. But the graph was blank.
I found myself surprisingly moved. It wasn’t just that I understood the elegance and depth of the achievement, and the scientific implications. I had been there in the days when LUX was making its careful way toward the Yates shaft, its fragile wires protected by the plodding pace, Gaitskell watching his cell phone. I had come to think of the detector as something more than machine, and science as akin to poetry. I had stood only a few feet away from LUX’s core, “the quietest verifiable place in the universe,” as Gaitskell said — not the world, the universe. I had come to think of radiation as noise and its absence as a thing of beauty, meticulously wrought.
Gaitskell described the radioactive quiet in LUX’s core this way: aboveground, the chaos of radiation is comparable to sound on the field at the beginning of the Super Bowl, when the entire crowd is clapping. Within LUX’s core, that noise has been reduced to one person clapping once a minute, and what the detector is seeking, out of those tiered and distant bleachers, is the sound of a single breath.
LUX had expanded the frontiers of silence, achieving an almost ultimate quiet in the place where all such notions of silence began, in the very spot where Ray Davis had installed his tank. In October, LUX began a second, much more extensive run of data collection, to be completed in 2016. Its successor, LUX-ZEPLIN, has received a funding commitment from the DOE and a British science organization, and will start collecting data by 2019. LUX-ZEPLIN will be twenty-seven times larger and one hundred times more sensitive than LUX. It will push dark-matter research to the point where we’re close to hearing something even fainter than a breath: the residual sweep of neutrinos from the Big Bang, like the movement of air inside a newborn baby’s lungs.
Ever since Galileo discovered Jupiter’s moons, the telescope has been the main instrument of astronomy, and symbolic of science in general. From Herschel’s hand-ground forty-eight-inch mirror to the two-hundred-inch Hale telescope to the ten-meter mirrors of today, the direction has always been more. The images we have received from telescopes are works of art, and the instrument itself an exquisite object, with its precise mirrors and lenses, the way it swings on its bearings to follow the obverse roll of the sky. But telescopes reach out and for. They negate, in an instant, a kind of privacy. I have seen people pull away from the eyepiece, as if that first, all-at-once view of a planet were too much to bear, like a person seen naked. With telescopes we focus, gaze, gather. And designed into them, too, is the notion that if only more can be collected, we will remove the cloak of distance and uncover the secrets of the world and control its consummate processes.
Underground labs, on the other hand, evoke a different set of metaphors. In explaining their value, everyone I spoke with used the word “quiet.” I began to think of neutrinos and dark matter as whispers: the most intimate messages of the universe’s voice, carrying its closest secrets to ears that are all but deaf — or, perhaps more accurately, immune, because so other-natured.
With Earth itself as part of the instrument, underground labs simply receive. Designed into them is the knowledge that everything floats on a sea. And then there is the tank of water, and deep within it, the core of transparent xenon. The method of an underground lab is less-from-too-much. One feels that lovely lessening in spite of all the money invested — science as introversion and withdrawal, setting up the conditions of silence and waiting for the smallest voice of the universe, the voice of its conception. It’s this poetry I appreciate, the womb of the universe in its dark bigness, its amniotic sea of particles touching that smaller womb we have recognized our tiny Earth to be.
Part of me is excited by the possibilities of neutrino and dark-matter research, the sci-fi glitz of better health and medicine, longer and richer lives, interstellar travel. Another, quieter part of me, though, wonders. What if we have arrived at knowledge that we cannot mine or turn into something — arsenic, dynamite, trucks — that helps us mine something else and in so doing produces, always, another thing we cannot get our minds around? What if dark matter and neutrinos are so out of reach that all we can do is think about them, not manipulate or change them or mix them into new combinations? Of the many revolutions science has offered us — and challenged us with — that could be the quietest and the largest and the most interesting of all.