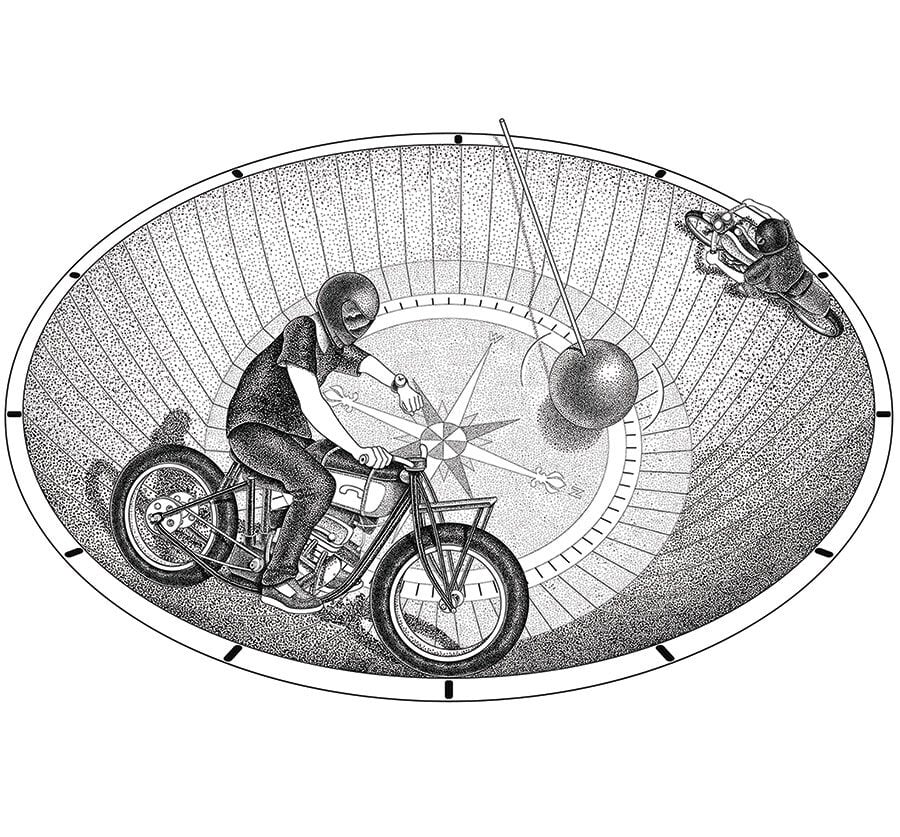
Illustrations by Dima Kashtalyan
When I was a kid, in the touch-tone era in the Midwest, I often dialed, for no real reason, the “time lady”—an actress named Jane Barbe, it turns out—who would announce, with prim authority “at the tone,” the correct time to the second. I was, in those days, a bit obsessed with time. I would stare, transfixed, at the Foucault pendulum at Chicago’s Museum of Science and Industry as it swept slow traces through its day; or gawp at the patinaed green clock, topped by a scythe and hourglass-carrying temporal patriarch and marked with a single word—